On May 3 the Department of Energy announced that two Fermilab scientists, Sam Posen and Jen Raaf, are recipients of the prestigious DOE Early Career Research Award.
Each will receive $2.5 million, distributed over five years, to advance their work at the laboratory.
Detailed detection for neutrinos
Raaf’s award will fund the construction of a detector that could help scientists better distinguish multiple, nearly simultaneous neutrino interactions from one another. It will also allow better visualization of neutrino interactions. The potential of this type of detector makes it a good candidate for the future international Deep Underground Neutrino Experiment, or DUNE.
Raaf builds on her experience as construction manager for the MicroBooNE detector and as co-spokesperson for the LArIAT neutrino R&D experiment. MicroBooNE, LArIAT and other upcoming Fermilab neutrino experiments use the detection technology planned for DUNE: a liquid-argon time projection chamber.
“Jen has a wide range of experience to draw upon to ensure the success of this experiment, and she knows how to get things done,” said Boston University physicist Ed Kearns, who is a member of the LArIAT and DUNE collaborations.
The advantage of the detector Raaf is developing lies in the fact that it will use argon not in its liquid form, but rather as a high-pressure gas.
When a neutrino sails into a sea of argon inside a detector and interacts with an argon nucleus, the interaction leaves behind a trail of ionized argon atoms, resulting in a track of electrons. The electron track drifts toward wires or pads — called a readout plane — inside the detector. The arrival of the electrons at the readout plane is like the arrival of particles of light at a camera sensor: The wires effectively take a picture of the electron track, translating the data into information about the neutrino that started it all.
Liquid-argon time projection chambers take exquisitely detailed three-dimensional images of particle interactions. But for an extremely intense neutrino beam, liquid argon might not cut it.
That’s because electrons drift through liquid argon relatively slowly. If neutrinos interact with argon at a rate faster than the wires can capture them — that is, faster than the time it takes for electrons to drift to the wires — multiple interactions could become indistinguishable. Scientists call this complication “pileup.”
Particles drifting through gaseous argon under high pressure, on the other hand, move much more quickly. So when neutrinos pour in, and two or more interact with gaseous argon in super-rapid succession, they won’t pile up on their way to the charged wires.
“You can better distinguish multiple events arriving close in time than you would in a liquid detector,” Raaf said. “So if you can make it easier for yourself to reconstruct the events, why not do that?”
Gaseous-argon detectors also afford scientists a more detailed look at what happens when a neutrino interacts with a nucleus. Because gaseous argon is less dense than liquid argon, low-energy particles resulting from the neutrino interaction travel farther, leaving behind a longer, more informative track.
“Neutrino experiments have been wrestling with what happens at the nucleus for some time, and the ArgoNeuT experiment at Fermilab has already shown surprising results about the emission of nucleons during neutrino interactions,” Kearns said. “By building a gaseous version of the liquid-argon detector, Jen’s detector should reveal more detail about what is going on.”
Scientists use gaseous-argon detectors in other particle physics projects, but this will be the first developed for a neutrino-focused experiment. Raaf’s prototype detector, about the size of a microwave, will reside in a refrigerator-sized vessel that will be pressurized with gaseous argon at up to 20 times normal atmospheric pressure. Over the next five years, her team will design, build and test the detector, both in Fermilab’s test beam and in the neutrino beam from the Fermilab Main Injector.
If tests are successful, not only would scientists use the detector to explore neutrino-nucleus interactions, but it could also be put forward as a candidate for the DUNE near detector, which will be built at Fermilab close to the neutrino source. (The DUNE far detector, a liquid-argon detector, will be built at the Sanford Underground Research Facility in South Dakota, 800 miles from Fermilab.)
“We invest in promising young researchers early in their careers to support lifelong discovery science to fuel the nation’s innovation system,” said Cherry Murray, director of the DOE Office of Science. “We are proud of the accomplishments these young scientists already have made and look forward to following their achievements in years to come.”
Superior superconductivity for acceleration
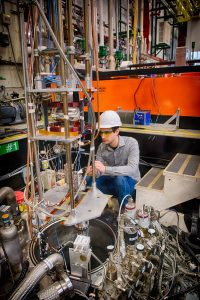
Sam Posen sets up a superconducting accelerating cavity in a vertical test stand. Photo: Reidar Hahn
Posen received his Early Career Research Award to develop a method for enhancing particle beam acceleration using niobium-tin, a material that has shown promise but whose full potential scientists are only beginning to investigate.
The application of a thin film of niobium-tin, or Nb3Sn, to accelerating cavities made of niobium could shorten the distance it takes for accelerators ramp up the energy of particle beams and decrease the cryogenic capacity required to keep the cavities cold. This would drive down the cost of accelerators not only for fundamental physics, but also for industry.
Superconducting accelerating cavities are traditionally made of niobium. The electromagnetic fields inside the cavities, which are shaped like strands of jumbo pearls and are arranged end to end in an accelerator, kick particle beams forward, accelerating them to increasingly high energies.
Scientists are working to improve accelerating cavities in two major areas. One is called quality factor, which is a measure of the cavity’s efficiency. The higher the quality factor, the more efficient the cavity. The second is accelerating gradient. The higher the cavity’s gradient, the more energy the particle beam gains over a given distance.
“We’re reaching close to the fundamental magnetic field limits of niobium, but for a very high-energy linear accelerator, it would still take thousands of cavities to reach the design energy,” Posen said. “If we can bypass this limit on the accelerating gradient, then we can cut that number down significantly and increase the reach of superconducting accelerators.”
One way to do that is to use a superior superconducting material.
“Niobium is a great material for cavities, but high-quality niobium-tin would be even better,” Posen said.
By applying 2 to 3 micrometers of niobium-tin to the cavities’ interior walls, scientists increase the flow of electric current through the cavities, strengthening the electromagnetic fields inside them and increasing the beam’s acceleration rate. That means particle accelerators could impart the desired beam energies using fewer cavities.
And whereas niobium has to be cooled to 2 Kelvin to achieve the quality factor that scientists are after for particle physics accelerators, niobium-tin can get to those high quality factors at warmer temperatures — roughly 4 Kelvin. The jump from 2 to 4 Kelvin may not seem big, but cooling infrastructure and costs go way down at the higher temperature. And for small-scale, industrial applications, 4-Kelvin operation opens up the possibility of using smaller, more affordable tabletop cryocoolers.
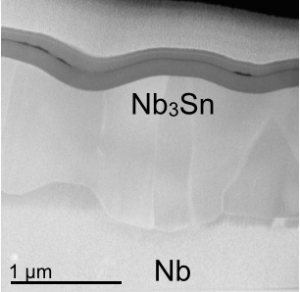
This is a transmission electron microscope image of a cross section from a niobium-tin layer on niobium bulk for use in superconducting radio-frequency accelerating cavities. Photo: Yulia Trenikhina, TD
With fewer cavities and higher operating temperatures, energy efficiency goes up, and the footprint of high-energy accelerators goes down significantly, as does the cost.
“Sam’s Ph.D. research at Cornell produced very nice and promising results, and that’s why we recruited him to Fermilab — we wanted him to extend his research to real, full-scale accelerator cavities in large, high-energy physics installations,” said Sergey Belomestnykh, Fermilab chief technology officer and head of its Technical Division. “His work could end up shrinking the size of large accelerators, and that could lead to big cost savings. It could also lead to mobile high-power devices for industry.”
The challenge is perfecting the method for coating cavity walls with niobium-tin. Posen’s team will build on previous work on a process called vapor diffusion. The niobium cavity and a nearby tin source are both placed in a furnace, which is heated to about 1,100 degrees Celsius, and the tin to about 1,200 degrees Celsius. The tin melts, and the resulting vapor alloys with the inside of the niobium cavity.
Posen’s team will research the optimum conditions — temperature, furnace time, pre- and post-furnace cavity treatment — that result in the best niobium tin coat, one with the right ratio of niobium and tin and free of defects.
The next five years will be spent investigating the process on small-scale R&D-type cavities, culminating in tests on full-scale, production cavities. These include types of cavities that will be used at Fermilab for a future high-power accelerator for neutrino experiments, such as the PIP-II accelerator, and cavities for the proposed International Linear Collider.